ATP & ADP
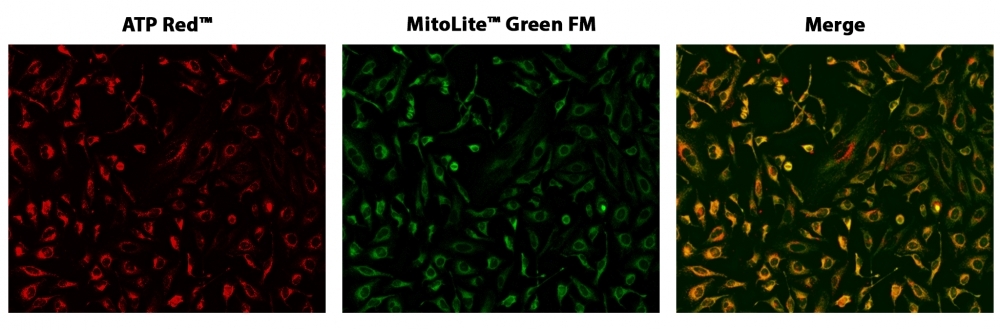
The fluorescence images of HeLa cells co-stained with ATP Red™ and MitoLite™ Green FM in a 96-well black-wall clear-bottom plate. Cells were washed twice with assay buffer before imaging. ATP Red™ and MitoLite ATP Red™ signals overlay well. (Far right image) The cells were imaged using a fluorescence microscope with a Cy3/TRITC and FITC filters.
Table of Contents
- ATP Function
- ATP and ADP Synthesis
- Quantification of ATP and ADP
- 3.1 Bioluminescence
- 3.2 Quantitative Nuclear magnetic Resonance (qNMR) Spectroscopy
- 3.3 High-performance liquid chromatography (HPLC) and LC mass spectrometry (LC-MS)
- 3.4 Respirometry
- 3.5 Chemiluminescence and Electrochemiluminescence (ECL) Biosensors
- 3.6 Förster Resonance Energy Transfer (FRET) Biosensors
- 3.7 Fluorescent Biosensors (Ratiometric and Intensiometric)
- Product Ordering Information
- References
When the bond between the second and third phosphate is broken, energy is released and ATP is converted to adenosine diphosphate (ADP). Hydrolysis frees these inorganic phosphate groups, which releases free energy (approximately ~7.3 cal/mol) that can be transferred to other molecules to make unfavorable reactions in a cell favorable. ADP can be powered back to ATP through a number of ways, namely aerobic respiration in the mitochondria or the photosynthetic pathways in plants. Likewise, energy is also released when another phosphate is removed from ADP to form adenosine monophosphate (AMP).
AMP also has the ability to recycle into ADP or ATP by forming new bonds to store potential energy. Normal intracellular concentration of ATP ranges between 1-10 uM, and the body depends upon the hydrolysis of 100-150 moles of ATP per day. This means that AMP, ADP, and ATP require constant interconversion in order to keep up with the energy demand of biological reactions.
ATP Function
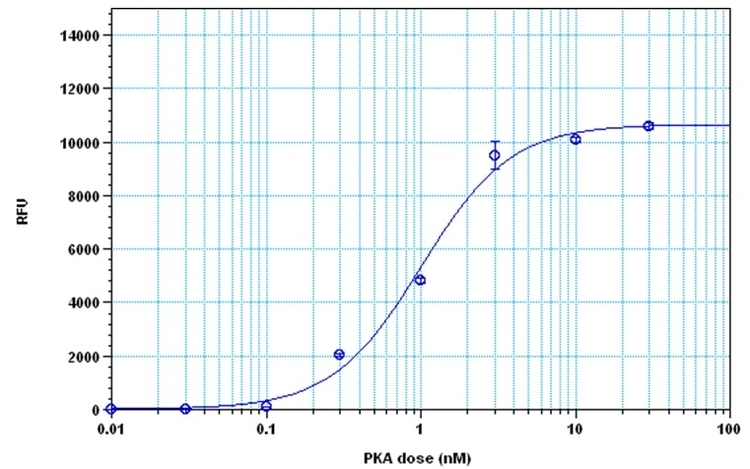
The detection of protein kinase A with PhosphoWorks™ Fluorimetric ADP Assay Kit. The kinase was incubated in the presence of ATP and kemptide peptide substrate for 30 minutes, and ADP generation was detected after 30 minutes incubation using the kit.
ATP also serves a purpose in signal transduction, which can utilize kinase phosphorylation of a protein to activate a signaling cascade. Here, ATP is used as a substrate for kinases, the most numerous ATP-binding protein. Mechanistically kinase is, in part, tightly regulated through magnesium ions complexed with ATP, bound at the phosphate-oxygen centers. Additionally ATP plays a critical role in active transport across the cell membrane of macromolecules in and out of the cell, known as endocytosis and exocytosis, respectively. In this process ATP hydrolysis provides the energy for active transport mechanisms to carry molecules like proteins and lipids across a concentration gradient. Using active transport, ATP can also pump calcium ions from the myoplasm and pull sodium and potassium ions from the sarcolemma against their concentration gradients.
ATP helps preserve cellular structure through the assembly of the cytoskeletal elements, and supplies energy to the flagella and chromosomes to maintain functionality. In muscle contraction, ATP generates force against adjoining actin filaments through the cycling of myosin cross-bridges. ATP and other purine nucleotides mediate extracellular paracrine signaling that controls autonomic functions, neural glial interactions, pain, and vessel tone.
Likewise, ATP plays a major role in neurotransmission. The brain is the highest consumer of ATP in the body, consuming roughly 25% percent of the total energy available. A lot of available free energy goes into maintaining ion concentrations for neuronal signaling and synaptic transmission. For this, ATP establishes ion gradients at the presynaptic terminal that shuttle neurotransmitters into vesicles and prime the vesicles for exocytotic release. Then, the action potential propagates through the axon via depolarization, sending a signal towards the axon terminal. Subsequently ATP must restore the ion concentration in the axon after each action potential, allowing another signal to occur. ATP is co-stored and co-released among neurotransmitters, in both sympathetic and parasympathetic nerves that help control neural and glial cells.
ATP is additionally an ubiquitous trigger of intracellular messengers for hormones, various enzymes, lipid mediators, nitric oxide, growth factors, and reactive oxygen species. Alternatively, ADP is a platelet agonist, is actively secreted from platelet dense granules, and is passively released from damaged erythrocytes and endothelial cells. ADP activates platelets via cell surface receptors coupled to guanosine triphosphate (GTP)-binding proteins.
Application Notes: |
ATP and ADP Synthesis
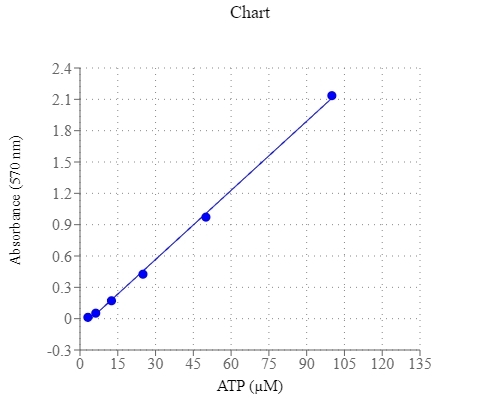
ATP dose response measured with PhosphoWorks™ Colorimetric ATP Assay Kit in a 96-well clear bottom plate using a SpectraMax microplate reader (Molecular Devices).
Table 1. Amplite® NAD/NADH Assay Kits
Cat# ▲ ▼ | Product Name ▲ ▼ | Ex ▲ ▼ | Em ▲ ▼ | Unit Size ▲ ▼ |
15257 | Amplite® Fluorimetric Total NAD and NADH Assay Kit *Red Fluorescence* | 540 | 590 | 400 Tests |
15258 | Amplite® Colorimetric Total NAD and NADH Assay Kit | 575 | 400 Tests | |
15261 | Amplite® Fluorimetric NADH Assay Kit *Red Fluorescence* | 540 | 590 | 400 Tests |
15263 | Amplite® Fluorimetric NAD/NADH Ratio Assay Kit *Red Fluorescence* | 540 | 590 | 250 Tests |
11321 | Amplite® Colorimetric Glyceraldehyde-3-Phosphate Dehydrogenase (GAPDH) Assay Kit | 450 | 100 Tests | |
15271 | Amplite® Colorimetric NADH Assay Kit | 460 | 400 Tests | |
15273 | Amplite® Colorimetric NAD/NADH Ratio Assay Kit | 460 | 250 Tests | |
15275 | Amplite® Colorimetric Total NAD and NADH Assay Kit *Enhanced Sensitivity* | 460 | 400 Tests | |
15280 | Amplite® Fluorimetric NAD Assay Kit *Blue Fluorescence* | 420 | 480 | 200 Tests |
Another method of ATP synthesis involves beta-oxidation. Throughout each cycle of beta-oxidation fatty acid chains are permanently shortened, reduced by two carbons, to produce one molecule of acetyl-CoA. Acetyl-CoA can be oxidized in the citric acid cycle, and form one molecule of NADH and FADH2. These molecules can transfer their high energy electrons to the electron transport chain, aiding in the generation of ATP. Moreover, ketosis and anaerobic respiration can generate ATP. During ketosis, ketone bodies undergo catabolism to generate twenty-two ATP molecules and two GTP molecules per acetoacetate molecule.
During anaerobic conditions where oxygen is scarce or unavailable, cells may opt to undergo anaerobic respiration. In this situation, there is a buildup of NADH since these molecules cannot oxidize to NAD+, limiting glucose consumption. To maintain homeostasis, pyruvate is reduced to lactate, yielding the oxidation of one NADH molecule in a process called lactic fermentation. This maintains the NAD+ reservoir, and produces two molecules of ATP for every molecule of glucose consumed.
Quantification of ATP and ADP
Bioluminescence
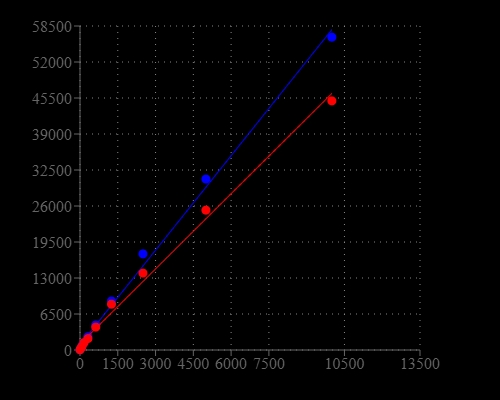
Cell number correlates with the luminescent signal. Different Jurkat cell number (2-fold dilution) was measured using the ReadiUse™ Rapid Luminometric ATP Assay Kit in a 96-well white plate. The kit can detect as low as 50 cells. There is a linear relationship (r2 >0.99) between the luminescent signal and cell number after 15 minutes or 2 hours incubation. The half-life of luminescent signal is more than 2 hours.
By adding a localization signal to the luciferase it also becomes possible to target cell subcompartments (e.g., mitochondria, cell membrane, or nucleus). Though widely commercially available, the luciferase assay does not come without consideration. Luciferase has poor specificity for ATP, and may produce ATP from the pool of ADP present in the sample. Luciferase activity can also be disturbed by cellular inhibitors or other cellular activities, which may reduce its capacity for bioluminescence.
Table 2. ATP assays for measuring cell proliferation.
Cat# ▲ ▼ | Product Name ▲ ▼ | Unit Size ▲ ▼ |
21601 | Readiuse™ Rapid Luminometric ATP Assay Kit | 100 Tests |
21602 | Readiuse™ Rapid Luminometric ATP Assay Kit | 1000 Tests |
21603 | Readiuse™ Rapid Luminometric ATP Assay Kit | 10,000 Tests |
21608 | PhosphoWorks™ Luminometric ATP Assay Kit *Extended Luminescence* | 10 Plates |
21609 | PhosphoWorks™ Luminometric ATP Assay Kit *Extended Luminescence* | 1 Plate |
21610 | PhosphoWorks™ Luminometric ATP Assay Kit *Maximized Luminescence* | 1 Plate |
21612 | PhosphoWorks™ Luminometric ATP Assay Kit *DTT-Free* | 1 Plate |
21613 | PhosphoWorks™ Luminometric ATP Assay Kit *DTT-Free* | 10 Plates |
21617 | PhosphoWorks™ Colorimetric ATP Assay Kit | 100 Tests |
21620 | PhosphoWorks™ Fluorimetric ATP Assay Kit | 100 Tests |
Quantitative Nuclear magnetic Resonance (qNMR) Spectroscopy
qNMR spectroscopy is based on the properties of specific atoms having an odd number of protons and/or neutrons. ATP, ADP, and AMP molecules contain a large proportion of hydrogen and potassium atoms, which mean they behave as magnetic dipoles. When these atoms are subjected to a magnetic field, they absorb an electromagnetic wave and emit a detectable energy peak, and the peak area is directly proportional to the number of H and P atoms present.
The main advantage of qNMR spectroscopy is that it is perfect linearity in between 0.1 to 100 mm for 1H-NMR, and 1-75 mm for 31P-NMR (where 1H and 31P are commonly used as known standards). This method, however, comes with some limitations. The detection range of this technique is lower than the concentration of physiological ATP present in cells and tissues, limiting its use in current biological research and development. qNMR spectroscopy also has a low temporal resolution and requires long processing times, typically around 14 hours. Although extremely precise, qNMR needs very expensive equipment and significant amounts of biological materials, as in total cell extracts, to provide reliable results.
High-performance liquid chromatography (HPLC) and LC mass spectrometry (LC-MS)
HPLC works by separating molecules within a sample according to key features like polarity. HPLC includes a mobile and stationary phases where the molecules in the sample separate due to their different affinities for the test system, known as the column. Molecules with more affinity will be retained, while the others will be eluted. Such molecules will exit the column at different times, based on affinity, and secondarily passing through a detector for analysis which generates a chromatogram. HPLC has been used to determine ATP, ADP, and AMP levels in plant primary cells as well as mammalian cell lines. It should be stated that HPLC requires large amounts of cells as well as the complete extraction of cell contents, which may lead to the loss of nucleotides due to hydrolysis.
HPLC is a faster technique, can be automated, is highly reproducible, and very accurate. LC-MS adds the ability to detect and identify molecules by measuring their mass. In MS, molecules are first ionized, then the ionized molecules acquire a specific speed, which directly depends on their mass (m) and charge (z). After, an ion detector individually identifies each molecule to generate a spectrum, and each molecule can be sorted according to their m/z ratios. LC-MS makes it possible to obtain ATP concentration simultaneously and quickly. Like HPLC, LC-MS and MS alone require expensive instruments and sample preparation can be lengthy.
Respirometry
Respirometry (sometimes called oxygraphy), is an indirect, qualitative, method to measure efficiency of mitochondrial ATP. Respirometry is convenient, inexpensive, and based on the fact that oxygen consumption can directly be linked to ATP production; more cellular ATP production means more oxygen consumed. Respirometry uses selective drugs and metabolites to activate and/or inhibit the activity of respiration, and then measures the corresponding flux of oxygen.
First, a sample is placed in a specific support that undergoes sequential injection of different drugs and metabolites (e.g., succinate, ADP, and cytochrome c). The drug injection mixture normally consists of oligomycin (an antibiotic that inhibits ATP synthase by limiting oxygen consumption), FCCP (a weak lipophilic acid that easily becomes protonated and deprotonated, thereby limiting the ability of ATP synthesis), and antimycin and rotenone (complexes that block electron transfer). Technologies using respirometry have successfully assessed ATP in isolated mitochondria, cultured cells, and various tissue samples.
Chemiluminescence and Electrochemiluminescence (ECL) Biosensors
Chemosensors are chemiluminescence tools that are based on the chemical properties of ATP, and are capable of carrying out covalent, electrostatic, and hydrogen bond-interactions with its substrates. Chemosensors have been created that allow excellent selectivity for ATP over ADP and AMP, and have provided researchers with the ability to monitor mitochondrial ATP fluctuations in live cells. Many chemosensors are single-wavelength, ratiometric, and require a spectrometer for ATP quantification. Possibly the widest variety of chemosensors have been derived from rhodamine, a small organic molecule that emits fluorescence due to its xanthene nucleus.
For example, the chemosensor Rh6G-NH-PBA was created that consisted of rhodamine 6G, a diethylenetriamine group, and a phenylboronic acid group (Zejun et al, 2019). In this experiment without the presence of ATP, the probe did not emit light due to a closed ring structure. In the presence of ATP, however, interactions between the chemosensor and ATP triggered the ring to open and free the light-emitting Rh6G, which could then be quantified. Though novel, biophysical properties of many chemiluminescent probes are still not fully known. This has severely limited their use in live-cell imaging due to potential aggregation, quenching, and low photosensitivity.
ECL is a highly sensitive technique for the quantification of ATP at nanomolar levels that utilizes electron transfer on the surface of an electrode. Some research technologies have developed ECL sensors, which turn on and off when ATP is bound and unbound, respectively (Liu et al., 2014). This method was based on the use of quantum dots (QDs), also termed artificial atoms, which are semiconductor nanostructures capable of collecting electrical charges and emitting light according to their size. QDs were attached to an electrode and linked to a DNA sequence. The DNA is partially complementary with an aptamer, a synthetic oligonucleotide capable of binding a specific ligand, which was in this case ATP. In quantification, the aptamer preferentially binded to the ATP molecule, when present, providing a detectable quantifiable signal.
Förster Resonance Energy Transfer (FRET) Biosensors
FRET microscopy relies on the transfer of energy between a donor and acceptor fluorescent pair. By studying conformational changes of a given protein, a number of FRET-based biosensors have been developed. In particular, the ATEAM biosensor has been widely adapted in research. One study used the ATEAM biosensors to monitor intracellular ATP in living HeLa cells, and was detectable in the cytoplasm, nucleus, and the mitochondria by integrating an organelle-specific localization signal (Imamura et al, 2009). Variations of the ATEAM biosensor have additionally expanded its use and ability in assessing ATP.
Table 3. Ordering Information For All FRET Building Blocks
Cat# ▲ ▼ | Product Name ▲ ▼ | Unit Size ▲ ▼ |
5000 | FMOC-Asp(EDANS)-OH *CAS 182253-73-2* | 100 mg |
5003 | FMOC-Asp(5/6-FAM)-OH | 100 mg |
5004 | FMOC-Asp(5-FAM)-OH | 100 mg |
5005 | FMOC-Asp(5/6-TAMRA)-OH | 100 mg |
5006 | FMOC-Asp(5-TAMRA)-OH | 100 mg |
5007 | FMOC-Asp(TF3)-OH | 100 mg |
5009 | FMOC-Glu(EDANS)-OH *CAS 193475-66-0* | 100 mg |
5012 | FMOC-Glu(5/6-FAM)-OH | 100 mg |
5013 | FMOC-Glu(5-FAM)-OH | 100 mg |
5014 | FMOC-Glu(5/6-TAMRA)-OH | 100 mg |
Fluorescent Biosensors (Ratiometric and Intensiometric)
Ratiometric and intensiometric biosensors are based on a single fluorescent protein that exhibits a fluorescence signal or an increase in fluorescence emission at a corresponding wavelength. This increase in fluorescence occurs only when the molecule of interest, in this case ATP, binds to the sensor which induces a conformational change. In these probes, the most common fluorophores are circularly permuted fluorescent proteins. Such fluorophores have their N- and C-termini directly fused with a peptide linker, which creates new terminal ends close to the chromophore. As a result, the slightest change in conformation is easily detected by manipulating the fluorescence properties.
Product Ordering Information
Table 4. Biochemical assays for measuring ATP activity, formation or depletion.
Assay ▲ ▼ | Ex/Abs (nm) ▲ ▼ | Em (nm) ▲ ▼ | Cutoff (nm)¹ ▲ ▼ | Microplate Type ▲ ▼ | Unit Size ▲ ▼ | Cat No. ▲ ▼ |
PhosphoWorks™ Fluorimetric ATP Assay Kit | 540 | 590 | 570 | Solid black | 100 tests | 21620 |
PhosphoWorks™ Colorimetric ATP Assay Kit | 570 | - | - | Clear bottom | 100 tests | 21617 |
PhosphoWorks™ Luminometric ATP Assay Kit *Maximized Luminescence* | - | - | - | Solid white | 1 plate | 21610 |
PhosphoWorks™ Luminometric ATP Assay Kit *Maximized Luminescence* | - | - | - | Solid white | 10 plate | 21621 |
Cell Meter™ Live Cell ATP Assay Kit | - | - | - | Black wall/clear bottom | 100 Tests | 23015 |
Table 5. Biochemical assays for measuring ADP activity, formation or depletion.
Assay ▲ ▼ | Ex (nm) ▲ ▼ | Em (nm) ▲ ▼ | Cutoff (nm)¹ ▲ ▼ | Microplate Type ▲ ▼ | Unit Size ▲ ▼ | Cat No. ▲ ▼ |
PhosphoWorks™ Fluorimetric ADP Assay Kit *Red Fluorescence* | 540 | 590 | 570 | Solid black | 100 tests | 21655 |