Basics of Biolayer Interferometry (BLI)
by Becky Graves, Jessica Piczon
Understanding Bio-Layer Interferometry: Principles, Comparison, & Applications
Biolayer Interferometry for Label-Free Biomolecular Interaction Analysis
Biolayer Interferometry (BLI) | The Biophysics behind the BLI Technology, Explained
Applications of Surface Plasmon Resonance and Biolayer Interferometry for Virus-Ligand Binding
Biolayer interferometry for DNA-protein interactions
High-throughput bio-layer interferometry is changing how new drugs are developed
Strategies Using Bio-Layer Interferometry Biosensor Technology for Vaccine Research and Development
Original created on March 14, 2024, last updated on March 14, 2024
Tagged under: protein, optics, antibodies, epitope, analysis
Biolayer Interferometry (BLI) is a relatively new optical technique that analyzes macromolecular interactions in real-time, producing more high-throughput information than previously designed technologies. By use of a simple, specific type of biosensor that is dipped directly into an analyte solution, researchers and scientists can track protein interactions and kinetics, label-free and with minimal signal noise, by assessing changes in the interference pattern of white light reflected from two different surfaces.
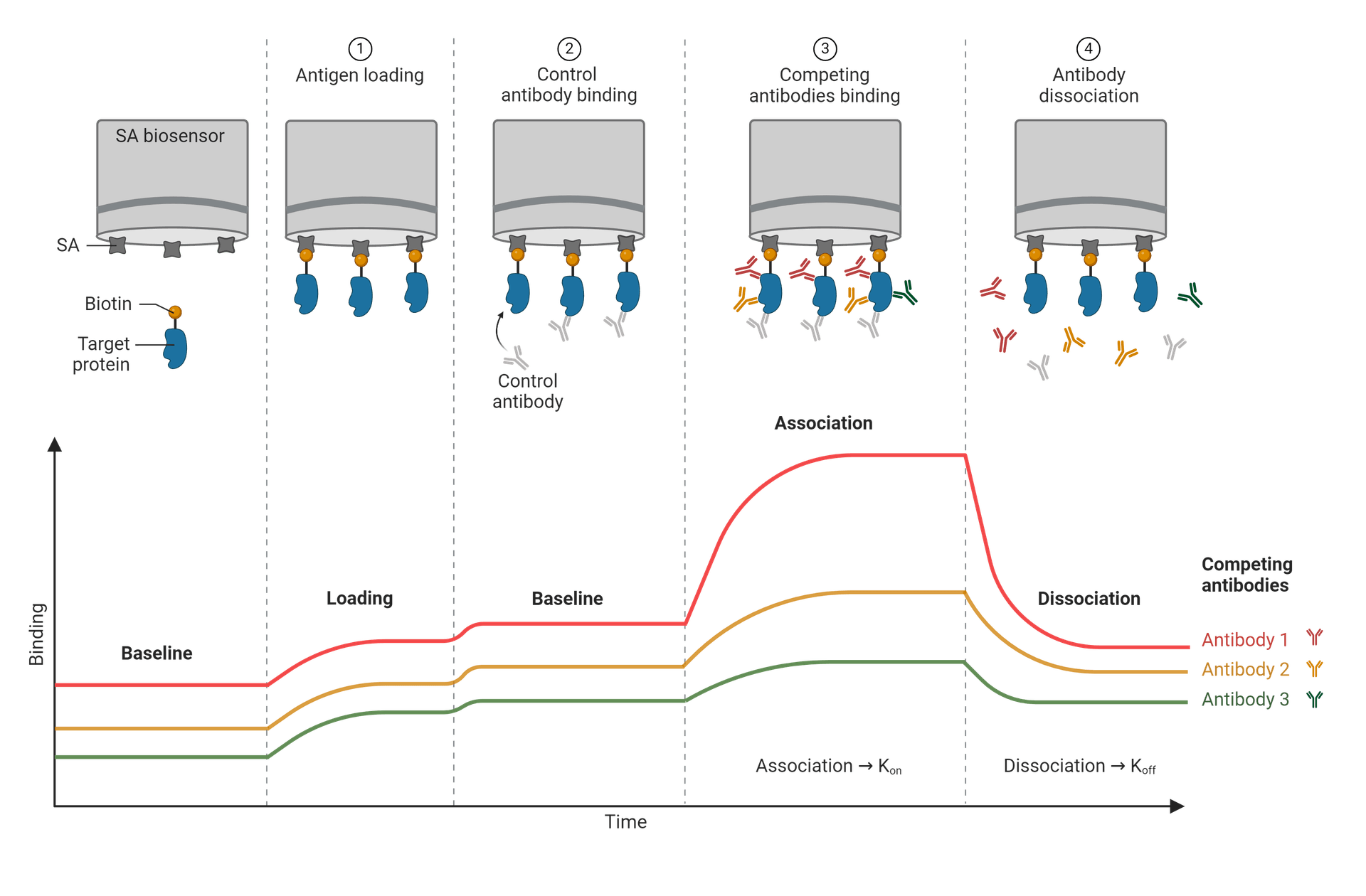
BLI comes as an improvement to a technique called surface plasmon resonance, or SPR. SPR and BLI are similar in that they can quantify molecular interaction data, but BLI is much more reliable, more cost-effective and is capable of allowing hundreds of interactions to occur at one time. In both SPR and BLI, a biomolecule acts as bait and others as the analytes, where a particular protein is isolated and immobilized. The biosensors used in BLI are comprised of tips that are coated with a compound that will bind to the biomolecules for immobilization and are administered via a method called dip-and-read. The dip-and-read format is done by use of a robot, in either 96 or 384-well plates, transporting the tips directly to the analyte solution. High-volume, quantitative research such as this, with unmatched precision, makes BLI a superior method in studying macromolecular interactions.
After the biosensor tip is inserted into the analyte solution, white light is reflected off two layers; one is an internal reference layer, and the other is the biocompatible immobilized protein layer at the tip of the biosensor. The immobilized protein layer on the biosensor varies widely, but common choices are nickel-charged tris-nitrilotriacetic (NTA), streptavidin (SA), or anti-human IgG Fc (AHC or AHQ). Anti-histidine tag or amine-reactive couplings have been gaining prominence.
How each of these surfaces reflects the light will determine the wavelength interference. Analyzing the shift in wavelength between the two separate surfaces on the biosensor enables researchers to determine specificities in binding, rates of association (Kon or Ka) and disassociation (Koff or Kd), as well as concentration levels of the analytes used. A distinct interference pattern is created by the way in which the molecules are bound, and with any change in the number of binding molecules at a given time. A wavelength shift that is positive tells us that there is an increase in association, thus the biolayer thickness increases. On the contrary, a negative shift implies more disassociation and a decrease in biolayer thickness. As the light passes through the changing biofilm thickness levels, the interference pattern is produced.
A response curve, or sensorgram, is generated from the data collected showing the relation of time vs response as the binding takes place. Depending on the molecule binding sites, a period of association occurs, until a level of equilibrium is reached. At this point, all recognition sites are in saturation with the analyte solution and are uniform. As the target molecules begin to unbind, the process of disassociation begins.
Further studies can be done on disassociation kinetics by flushing the buffers to unbind the associated molecules and prep for added testing. There are a wide variety of possible buffers that are compatible with BLI, and are chosen based on what the sensor proteins will tolerate well. A base of PBS with added detergents (to minimize nonspecific binding) as well as other components is a basic common choice.
A basic protocol for performing biolayer interferometry requires a few principal guidelines, beginning by establishing a baseline wavelength before any biomolecules are introduced. Applying the BLI buffer solution to the biosensor before the addition of other biomolecules will determine the standard wavelength in which to compare to.
Once the molecule of interest is inserted for immobilization, the chosen analytes are then added, beginning the binding and association process. Any shifts in wavelength that are observed from this point can be measured against the predetermined baseline. The white light interference pattern will adjust based on the binding activity until disassociation begins and the bound analytes are then washed away by the addition of a secondary BLI buffer. This should be the same type of buffer solution used for the baseline measurement. If there were any corrections needed, then the same vial of buffer should be used to minimize inconsistencies.
A few BLI interactions include protein-protein, protein-small molecule, and DNA-protein. Protein-protein interactions provide insight into how protein complexes are assembled and sequenced, while small molecules bound with proteins can aid in the comprehension of drug mechanics. DNA-protein interaction studies are vital to understanding overall biological functions, including DNA replication and repair. Applications of BLI in molecular interaction analytics can be found in pharmaceutical and academic research, and more extensive kinetic studies. BLI innovation has led to the engineering of antibodies and to drug discoveries, advancements in immunology, virology, genetics and more.
Biolayer interferometry fills a bioanalytical need to determine binding parameters that will largely continue to advance our studies in life-science research.
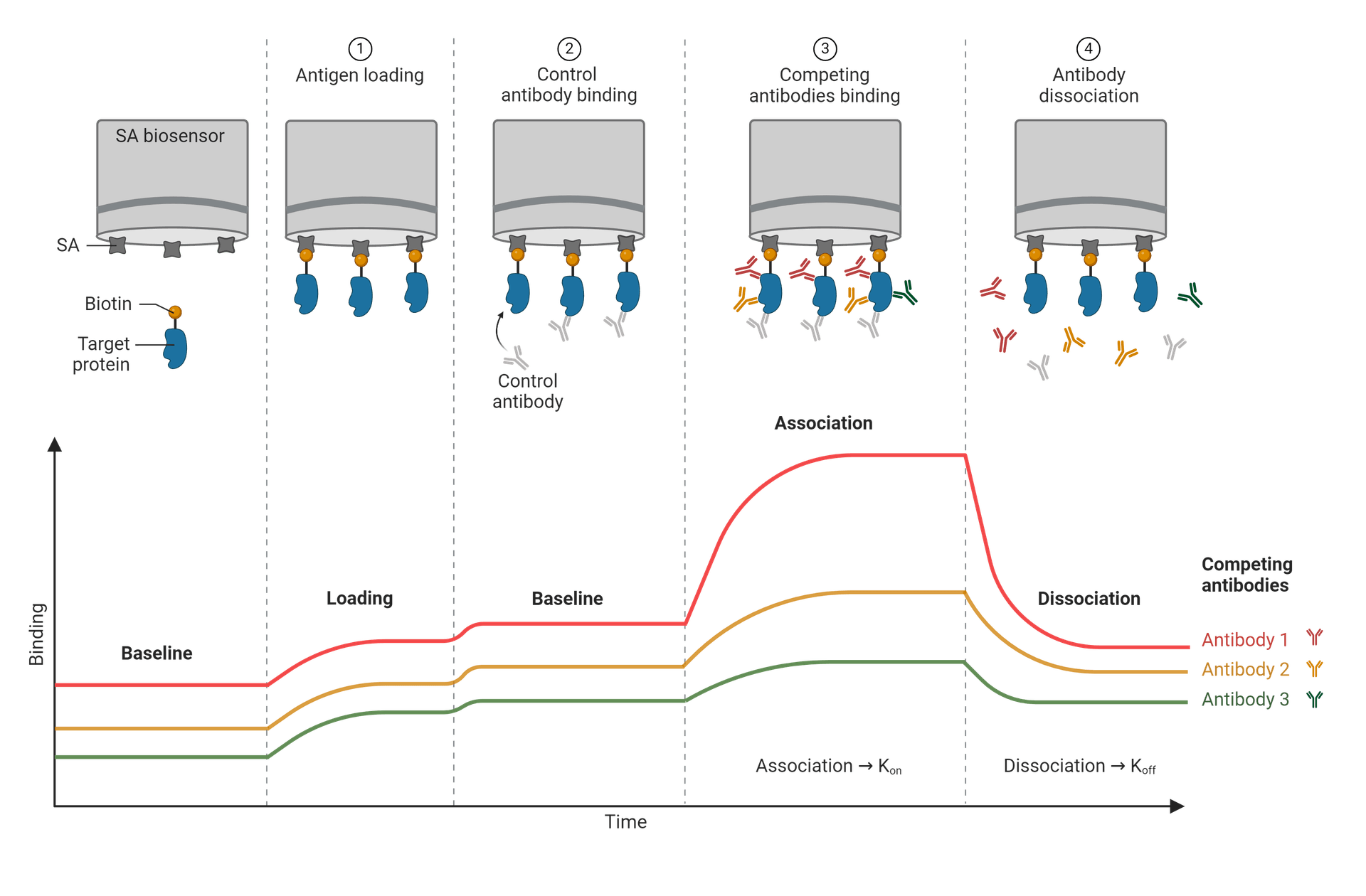
Basic steps of BLI along with the associated stages of the response curve. Each distinct line represents a different antibody epitope. In this example, the biosensor uses streptavidin (SA), and so the other steps will include biotinylated ligands, to take advantage of the strong streptavidin-biotin binding affinity. Figure made in BioRender.
After the biosensor tip is inserted into the analyte solution, white light is reflected off two layers; one is an internal reference layer, and the other is the biocompatible immobilized protein layer at the tip of the biosensor. The immobilized protein layer on the biosensor varies widely, but common choices are nickel-charged tris-nitrilotriacetic (NTA), streptavidin (SA), or anti-human IgG Fc (AHC or AHQ). Anti-histidine tag or amine-reactive couplings have been gaining prominence.
How each of these surfaces reflects the light will determine the wavelength interference. Analyzing the shift in wavelength between the two separate surfaces on the biosensor enables researchers to determine specificities in binding, rates of association (Kon or Ka) and disassociation (Koff or Kd), as well as concentration levels of the analytes used. A distinct interference pattern is created by the way in which the molecules are bound, and with any change in the number of binding molecules at a given time. A wavelength shift that is positive tells us that there is an increase in association, thus the biolayer thickness increases. On the contrary, a negative shift implies more disassociation and a decrease in biolayer thickness. As the light passes through the changing biofilm thickness levels, the interference pattern is produced.
A response curve, or sensorgram, is generated from the data collected showing the relation of time vs response as the binding takes place. Depending on the molecule binding sites, a period of association occurs, until a level of equilibrium is reached. At this point, all recognition sites are in saturation with the analyte solution and are uniform. As the target molecules begin to unbind, the process of disassociation begins.
Further studies can be done on disassociation kinetics by flushing the buffers to unbind the associated molecules and prep for added testing. There are a wide variety of possible buffers that are compatible with BLI, and are chosen based on what the sensor proteins will tolerate well. A base of PBS with added detergents (to minimize nonspecific binding) as well as other components is a basic common choice.
Tools: |
A basic protocol for performing biolayer interferometry requires a few principal guidelines, beginning by establishing a baseline wavelength before any biomolecules are introduced. Applying the BLI buffer solution to the biosensor before the addition of other biomolecules will determine the standard wavelength in which to compare to.
Once the molecule of interest is inserted for immobilization, the chosen analytes are then added, beginning the binding and association process. Any shifts in wavelength that are observed from this point can be measured against the predetermined baseline. The white light interference pattern will adjust based on the binding activity until disassociation begins and the bound analytes are then washed away by the addition of a secondary BLI buffer. This should be the same type of buffer solution used for the baseline measurement. If there were any corrections needed, then the same vial of buffer should be used to minimize inconsistencies.
A few BLI interactions include protein-protein, protein-small molecule, and DNA-protein. Protein-protein interactions provide insight into how protein complexes are assembled and sequenced, while small molecules bound with proteins can aid in the comprehension of drug mechanics. DNA-protein interaction studies are vital to understanding overall biological functions, including DNA replication and repair. Applications of BLI in molecular interaction analytics can be found in pharmaceutical and academic research, and more extensive kinetic studies. BLI innovation has led to the engineering of antibodies and to drug discoveries, advancements in immunology, virology, genetics and more.
Biolayer interferometry fills a bioanalytical need to determine binding parameters that will largely continue to advance our studies in life-science research.
Tools: |
Products
Table 1. Available Reactive Biotinylation Reagents
Product ▲ ▼ | Chemical Reactivity ▲ ▼ | Reactive Moiety ▲ ▼ | Unit Size ▲ ▼ | Cat No. ▲ ▼ |
Biotin, succinimidyl ester *CAS 35013-72-0* | Amine | Succinimidyl Ester | 100 mg | 3002 |
Biotin-X, succinimidyl ester *CAS 72040-63-2* | Amine | Succinimidyl Ester | 25 mg | 3010 |
Biotin PEG2 succinimidyl ester | Amine | Succinimidyl Ester | 25 mg | 3016 |
Biotin PEG4 succinimidyl ester | Amine | Succinimidyl Ester | 25 mg | 3022 |
Biotin C2 maleimide | Thiol | Maleimide | 25 mg | 3005 |
Biotin PEG2 maleimide *CAS 305372-39-8* | Thiol | Maleimide | 5 mg | 3015 |
Biotin PEG2 amine *CAS 138529-46-1* | Aldehyde, Carboxylic Acid, Ketone | Amine | 5 mg | 3014 |
Biotin PEG3 amine | Aldehyde, Carboxylic Acid, Ketone | Amine | 5 mg | 3024 |
Biotin cadaverine | Aldehyde, Carboxylic Acid, Ketone | Cadaverine | 5 mg | 3004 |
Biotin ethylenediamine *CAS 216299-38-6* | Aldehyde, Carboxylic Acid, Ketone | Ethylenediamine | 5 mg | 3003 |
Table 2. HIS Lite™ NTA-Ni conjugates
Cat# ▲ ▼ | Product Name ▲ ▼ | Unit Size ▲ ▼ |
12610 | HIS Lite™ Cy3 Bis NTA-Ni Complex | 1 mg |
12613 | HIS Lite™ Cy5 Bis NTA-Ni Complex | 1 mg |
12615 | HIS Lite™ OG488-Tris NTA-Ni Complex | 100 µg |
12617 | HIS Lite™ iFluor® 568 Tris NTA-Ni Complex | 100 µg |
12618 | HIS Lite™ iFluor® 647 Tris NTA-Ni Complex | 100 µg |
12619 | HIS Lite™ Cy5 Tris NTA-Ni Complex | 100 µg |
12620 | HIS Lite™ Cy3 Tris NTA-Ni Complex | 100 µg |
12621 | HIS Lite™ Cy3B Tris NTA-Ni Complex | 100 µg |
12650 | HIS Lite™ Cy3 Bis NTA Chelator | 100 µg |
12653 | HIS Lite™ Cy5 Bis NTA Chelator | 100 µg |
Table 3. Anti-human secondary antibodies.
Product ▲ ▼ | Host ▲ ▼ | Target Species ▲ ▼ | Conjugate ▲ ▼ | Ex (nm) ▲ ▼ | Em (nm) ▲ ▼ | Spectrum ▲ ▼ | Application ▲ ▼ | Size ▲ ▼ | Cat No. ▲ ▼ |
Purified goat anti-human IgG Fc (H+L) | Goat | Human | Unconjugated | WB, IHC/ICC/IF, ELISA, Flow | 1 mg | 50273 | |||
Purified goat anti-human IgG (H+L) | Goat | Human | Unconjugated | WB, IHC/ICC/IF, ELISA, Flow | 1 mg | 50269 | |||
APC goat anti-human IgG (H+L) | Goat | Human | APC | 651 | 660 | WB, IHC/ICC/IF, ELISA, Flow | 200 µg | 50184 | |
APC goat anti-human IgG (H+L) | Goat | Human | APC | 651 | 660 | Flow | 1 mg | 50185 | |
APC goat anti-human IgG (H+L) *Cross-adsorbed* | Goat | Human | APC | 651 | 660 | Flow | 200 µg | 50186 | |
APC goat anti-human IgG (H+L) *Cross-adsorbed* | Goat | Human | APC | 651 | 660 | Flow | 1 mg | 50187 | |
APC/Cy7 goat anti-human IgG (H+L) | Goat | Human | APC/Cy7 | 651 | 779 | Flow | 200 µg | 50188 | |
APC/Cy7 goat anti-human IgG (H+L) | Goat | Human | APC/Cy7 | 651 | 779 | Flow | 1 mg | 50189 | |
APC/Cy7 goat anti-human IgG (H+L) *Cross-adsorbed* | Goat | Human | APC/Cy7 | 651 | 779 | Flow | 200 µg | 50190 | |
APC/Cy7 goat anti-human IgG (H+L) *Cross-adsorbed* | Goat | Human | APC/Cy7 | 651 | 779 | Flow | 1 mg | 50191 |
Table 4. Streptavidin Products
Cat# ▲ ▼ | Product Name ▲ ▼ | Unit Size ▲ ▼ |
16900 | RPE-streptavidin conjugate | 100 µg |
16901 | RPE-streptavidin conjugate | 1 mg |
16902 | APC-streptavidin conjugate | 100 µg |
16905 | PerCP-streptavidin conjugate | 100 µg |
16906 | RPE-iFluor® 647-streptavidin conjugate | 100 µg |
16907 | RPE-iFluor® 750-streptavidin conjugate | 100 µg |
16908 | APC-iFluor® 750-streptavidin conjugate | 100 µg |
16910 | FITC-streptavidin conjugate | 1 mg |
16911 | Texas Red®-streptavidin conjugate | 1 mg |
16912 | Cy3®-streptavidin conjugate | 1 mg |
References
Understanding Bio-Layer Interferometry: Principles, Comparison, & Applications
Biolayer Interferometry for Label-Free Biomolecular Interaction Analysis
Biolayer Interferometry (BLI) | The Biophysics behind the BLI Technology, Explained
Applications of Surface Plasmon Resonance and Biolayer Interferometry for Virus-Ligand Binding
Biolayer interferometry for DNA-protein interactions
High-throughput bio-layer interferometry is changing how new drugs are developed
Strategies Using Bio-Layer Interferometry Biosensor Technology for Vaccine Research and Development
Original created on March 14, 2024, last updated on March 14, 2024
Tagged under: protein, optics, antibodies, epitope, analysis